dati bibliografici in fase di sviluppo.......STIAMO CARICANDO TUTTI I FILE ......SUL NUOVO SITO
- Laura Bertolotti Colombi
- 31 gen 2020
- Tempo di lettura: 17 min
Aggiornamento: 5 nov 2021
A specific mutation in TBL1XR1 causes Pierpont syndrome
Charlotte A Heinen,1,2 Aldo Jongejan,3 Peter J Watson,4 Bert Redeker,5 Anita Boelen,1 Olga Boudzovitch-Surovtseva,1 Francesca Forzano,6 Roel Hordijk,7 Richard Kelley,8 Ann H Olney,9 Mary Ella Pierpont,10 G Bradley Schaefer,11 Fiona Stewart,12 A S Paul van Trotsenburg,2 Eric Fliers,1 John W R Schwabe,4 Raoul C Hennekam13
ABSTRACT
Background
The combination of developmental delay, facial characteristics, hearing loss and abnormal fat distribution in the distal limbs is known as Pierpont syndrome. The aim of the present study was to detect and study the cause of Pierpont syndrome.
Methods
We used whole-exome sequencing to analyse four unrelated individuals with Pierpont syndrome, and Sanger sequencing in two other unrelated affected individuals. Expression of mRNA of the wild-type candidate gene was analysed in human postmortem brain specimens, adipose tissue, muscle and liver. Expression of RNA in lymphocytes in patients and controls was additionally analysed. The variant protein was expressed in, and purified from, HEK293 cells to assess its effect on protein folding and function.
Results
We identified a single heterozygous missense variant, c.1337A>C (p.Tyr446Cys), in transducin β-like 1 X-linked receptor 1 (TBL1XR1) as disease-causing in all patients. TBL1XR1 mRNA expression was demonstrated in pituitary, hypothalamus, white and brown adipose tissue, muscle and liver. mRNA expression is lower in lymphocytes of two patients compared with the four controls. The mutant TBL1XR1 protein assembled correctly into the nuclear receptor corepressor (NCoR)/ silencing mediator for retinoid and thyroid receptors (SMRT) complex, suggesting a dominant-negative mechanism. This contrasts with loss-of-function germline TBL1XR1 deletions and other TBL1XR1 mutations that have been implicated in autism. However, autism is not present in individuals with Pierpont syndrome.
Conclusions
This study identifies a specific TBL1XR1 mutation as the cause of Pierpont syndrome. Deletions and other mutations in TBL1XR1 can cause autism. The marked differences between Pierpont patients with the p.Tyr446Cys mutation and individuals with other mutations and whole gene deletions indicate a specific, but as yet unknown, disease mechanism of the TBL1XR1 p.Tyr446Cys mutation.
INTRODUCTION
In 1998 Pierpont and coworkers described two unrelated boys with remarkably similar faces (high forehead, underdeveloped mid-face, narrow palpebral fissures and anteverted nares), short stature, hearing loss, developmental delay and distinctive palmar and plantar fat pads.1 Two similar patients were subsequently reported, including one with a choroid plexus papilloma, whereupon the condition was named Pierpont syndrome.2 3 While several patients resembling Pierpont syndrome have been reported,4 clinical re-evaluation and molecular analyses have shown that they had either Coffin–Siris5 or Wiedemann–Steiner syndrome (H. Brunner, personal communication 2013). Initially this caused uncertainty about the phenotype defining Pierpont syndrome, but the characteristics of both Wiedemann–Steiner syndrome and Coffin–Siris syndrome are better known nowadays and allow easy differentiation from the phenotype in the reported patients with Pierpont syndrome.2 3 Until now, the cause of Pierpont syndrome has remained unknown. However, de novo autosomaldominant mutations were suspected to be the most likely cause.2 For the present study, we collected DNA from two newly identified and four earlier-reported patients with Pierpont syndrome. We performed whole-exome sequencing in four patients and in the parents of one of them, and identified identical de novo missense mutations in the transducin β-like 1 X-linked receptor 1 (TBL1XR1) gene in all four patients. Sanger sequencing of the two newly diagnosed patients revealed the same mutation. TBL1XR1 is part of the nuclear receptor corepressor (NCoR) complex that plays an essential role in gene transcription.
METHODS
Study cohort
The study cohort consisted of six unrelated patients with Pierpont syndrome (table 1). The clinical diagnosis was based on the combination of the facial characteristics, palmar and plantar fat pads, and global developmental delay (figure 1). The description of patients 1–4 has been published.1–3 Blood samples were obtained from all patients and their (unaffected) parents. Informed consent for the study was obtained from the parents of all patients. The Medical Ethics Committee of the Academic Medical Centre in Amsterdam (NL45117.018.13) approved the study.
Molecular analysis
Targeted enrichment and massive parallel sequencing were performed on genomic DNA extracted from circulating leucocytes of four patients and the Open Access Scan to access more free content 330 Heinen CA, et al. J Med Genet 2016;53:330–337. doi:10.1136/jmedgenet-2015-103233 Developmental defects on February 17, 2021 by guest. Protected by copyright. http://jmg.bmj.com/ J Med Genet: first published as 10.1136/jmedgenet-2015-103233 on 14 January 2016. Downloaded from parents of one of them. Enrichment of the whole exome was performed using the Nimblegen SeqCap EZ Library V.3.0 (Roche). Each captured library was then loaded on a SOLiD5500xl platform (Applied Biosystems) (patient 20120174 and unaffected parents 20112227 and 20112228, and patients 20112226, 20121069 and 20121072). Paired-end and singleend sequence reads were aligned to hg19 using the Lifescope aligner (V.2.5.1) (Applied Biosystems). Presumed PCR duplicates were discarded using Picard Tools (http://picard.sourceforge.net) in case of single-end reads or Lifescope when dealing with paired-end reads. Local realignment and base-quality-score recalibration were performed with the Genome Analysis Toolkit (GATK2 V.2.2-5-g3bf5e3f ).6
For reads mapping to the human genome reference, mean target region coverage for 20112226 and the trio was 94.5%, with average sequencing depth on target of 92× and for samples 20121069 and 20121072, respectively, 90.5% and 69×. Calls of SNPs and small insertions and deletions (INDELs) were based on 18 unrelated exomes using the GATK Unified Genotyper algorithm, and categorised based on their matching quality, depth of coverage, base quality, the combination of base quality and depth, the position of the alternate allele in the read, and strand bias.7 8 Variants were functionally annotated using KGGSeq v0.4 applying available public datasets from the 1000 Genomes Project, NHLBI GO Exome Sequencing Project and dbSNP (V.137), and predictions were made regarding probabilities of being disease causing. Only variants passing all applied GATK filters, predicted to be a de novo mutation within the trio and disease-causing by KGGSeq were retained.
Protein characterisation
The structure of the WD40 domain of TBL1XR1 was obtained from the Protein Data Bank (PDB accession code 4LG9). For expression in mammalian cells, constructs of TBL1XR1, HDAC3 and GPS2-SMRT chimaera were cloned into the pcDNA3 vector. Transient transfections and protein purifications were performed as described elsewhere.9 For the TBL1XR1/HDAC3/GPS2-SMRT chimaera complex, the GPS2-SMRT chimaera contained an N-terminal 10×His-3×Flag tag and a tobacco etch virus (TEV) protease cleavage site. HEK293F cells (Invitrogen) were cotransfected with mixtures of both tagged and untagged constructs using polyethylenimine (PEI) (Sigma). To transfect 60 mL of cells, 60 mg DNA total was diluted in 6 mL of phosphate-buffered saline (PBS) (Sigma) and vortexed briefly; 240 mL of 0.5 mg/mL PEI was added, then vortexed briefly, and incubated for 20 min at room temperature, then added to 60 mL cells (final density 1×106 cells/mL). Cells were harvested 48 h after transfection. For the interaction studies the cells were lysed by sonication in 50 mM Tris/Cl pH 7.5, 100 mM potassium acetate, 5% v/v glycerol, 0.3% v/v
Table 1 Main clinical features of presently described individuals with Pierpont syndrome, including updates of the published patients 1 and 2,1 32 and 43 Patient 1 2 3 4 5 6 Total Age (years) 28 20 12 5.7 10 19 Gender M M M M F F 4M/2F Growth parameters at birth* Length (cm) 45.7 (C in TBL1XR1 located at 3q26.32, resulting in the amino acid substitution p.Tyr446Cys (Y446C) in all four patients studied, but not in the parents of one of them (see online supplementary table S1). No other potentially pathogenic variant in the same gene was present in all four patients. Sanger sequencing demonstrated the same mutation in the two other patients. All other parents tested negative for the mutation using Sanger sequencing, indicating de novo occurrence. The mutation was at an evolutionary conserved position (figure 2A) and absent in control populations (dbSNP, 1000 genomes, NHBLI, ESP, GoNL). Stability of mutated complexes TBL1XR1 is a highly conserved protein found in all eukaryotes (see online supplementary figure S1). It contains two structured domains: an amino-terminal domain that mediates tetramerisation of the protein and a carboxy-terminal WD40 domain. The TBL1XR1 tyrosine-to-cysteine mutation identified here is located in the WD40 domain on one side of the inner surface of the WD40 ring (figure 2A). Y446 is largely exposed to solvent, and mutation to cysteine would not be expected to significantly perturb the structure of the domain. A tyrosine in this position is found in nearly all TBL1XR1 proteins, although the equivalent residue is a phenylalanine in the homologous Sif2 protein from Saccharomyces cerevisiae. The structure of the WD40 domain from Sif2 has also been reported.12 Despite relatively low sequence identity between TBL1XR1 and Sif2, the structures of their WD40 rings itself are similar. Furthermore, the yeast residue equivalent to Y446, f446, adopts a very similar conformation (figure 2B) suggesting a conserved function for this largely non-polar amino acid. To confirm that the Y446C mutation does not grossly perturb the fold and behaviour of TBL1XR1 we examined the ability of the TBL1XR1 to assemble correctly with the GPS2:SMRT:HDAC3 complex. As predicted, the mutant protein was readily expressed and purified and assembled correctly into the TBL1XR1:GPS2:SMRT:HDAC3 complex (figure 2C and D), suggesting that the molecular pathology of the Y446C mutation is an impaired protein–protein interaction with an as yet unidentified molecular partner rather than a failure to fold correctly.
mRNA expression
TBL1XR1 mRNA expression was well visible in the pituitary and the PVN area of the hypothalamus, as well as in liver and muscle tissue and both white and brown adipose tissue, fitting the clinical symptomatology of Pierpont syndrome (figure 3A, B). Figure 3 (A) Transducin β-like 1 X-linked receptor 1 (TBL1XR1) mRNA expression in human pituitary and hypothalamic PVN. (B) TBL1XR1 mRNA expression in human white and brown adipose tissue, liver and muscle tissue. TBL1XR1 transcript PCR product on 2% agarose gel. The expected product is 126 bp. BAT, brown adipose tissue; PIT, pituitary; PVN, paraventricular nucleus; WAT, white adipose tissue. Figure 4 Relative expression of transducin β-like 1 X-linked receptor 1 (TBL1XR1) mRNA to hypoxanthine phosphoribosyl transferase (used as reference gene) in leucocytes of patients (closed circles) and controls (open circles). Individual values are depicted and mean values ±SD is represented by a solid line. 334 Heinen CA, et al. J Med Genet 2016;53:330–337. doi:10.1136/jmedgenet-2015-103233 Developmental defects on February 17, 2021 by guest. Protected by copyright. http://jmg.bmj.com/ J Med Genet: first published as 10.1136/jmedgenet-2015-103233 on 14 January 2016. Downloaded from TBL1XR1 mRNA expression in whole blood was lower in patients compared with controls (figure 4). The small number of patients available for analysis precludes statistical analysis to determine whether this difference is significant. DISCUSSION In this study, we found a single TBL1XR1 missense mutation in six patients with Pierpont syndrome that was absent in their unaffected parents. TBL1XR1, a member of the WD40 repeat-containing protein family, is composed of 18 exons.13 The product of TBL1XR1, TBL1XR1 (or TBLR1; 55 595 Da; 514 amino acids), contains a carboxy-terminal WD40 domain containing eight WD40 repeats and an amino-terminal LisH domain that mediates tetramerisation of the protein and its interactions with NCoR/SMRT and GPS2.13 14 TBL1XR1 is an essential component of the NCoR/SMRT corepressor complex (figure 2C), which interacts with nuclear hormone receptors, a family of ligand-dependent transcription factors involved in regulation of gene transcription (figure 5).15 When bound to unliganded nuclear hormone receptors, corepressors mediate silencing of gene transcription by recruiting chromatin-modifying enzymes. When a nuclear hormone receptor is liganded, corepressors dissociate to relieve repression of transcription. In negatively regulated target genes, corepressors are essential for activation of transcription.16 The WD40 repeats in TBL1XR1 are thought to be involved in the interaction of the corepressor complex with histones stabilising the association of the complex with the chromatin.13 17 TBL1XR1 also may play a regulatory role in the NF-κB pathway and Wnt-mediated transcription. NF-kB transcription requires IKKα to phosphorylate SMRT on chromatin, which recruits TBL1XR1 to the gene promoter. During depletion of TBL1XR1, NF-kB transcription and cell survival are compromised.17 18 TBL1XR1 also recruits β-catenin to the Wnt targetgene promoter. In the presence of TBL1XR1, β-catenin is able to remove corepressors from the promoter of Wnt target genes by competitive binding, thereby activating transcription.19 TBL1XR1 mutations have been implicated in several phenotypes. Recurrent TBL1XR1 mutations have been described in DNA of lymphatic malignancies, including primary central nervous system lymphomas,20 21 acute lymphoblastic leukaemia22 and Sézary syndrome.23 The precise mechanisms by which TBL1XR1 mutations contribute to tumourigenesis remain unclear, but it has been hypothesised that loss of TBL1XR1 could compromise the ability of corepressor complexes to inhibit receptor activity, leading to increased activation of receptor target genes.22 In addition, it has been suggested that TBL1XR1 might act as a tumour-suppressor gene in the lymphatic system,21 and upregulates VEGF-C inducing lymphangiogenesis in oesophageal squamous cell carcinoma.24 Contrarily, TBL1XR1 has been nominated as a novel breast cancer oncogene,25 indicating that any role of TBL1XR1 in tumourigenesis is tissue-specific. Whole-exome sequencing of 209 children with autism spectrum disorder (ASD) and their parents showed a de novo p.Leu282Pro mutation in TBL1XR1 in one child, who also had intellectual disability.26 Subsequently, evaluation of 44 candidate genes in 2494 ASD cases identified two de novo TBL1XR1 mutations (p.Leu282Pro, p.Ile397SerfsX19).27 Apart from intellectual disability, however, these patients had no features in common with Pierpont syndrome (B O’Roak and E Eichler, personal communication 2013). Similarly, a non-dysmorphic patient with intellectual disability, autism and West syndrome was found to have a de novo p.Gly70Asp mutation in TBL1XR1.28 Three patients, including a mother and child, have been described with a deletion involving only TBL1XR1 in patients who had mild to moderate intellectual disability without autistic behaviour or manifestations of Pierpont syndrome.29 30 In addition, three cases with small deletions involving TBL1XR1 and other genes are included in the Decipher database (http://decipher.sanger.ac.uk/). All had intellectual disability, ASD or ASD-like behaviour but lacked physical signs of Figure 5 Schematic model of transcriptional regulation by the SMRT/ nuclear receptor corepressor (NCoR) complex. Heinen CA, et al. J Med Genet 2016;53:330–337. doi:10.1136/jmedgenet-2015-103233 335 Developmental defects on February 17, 2021 by guest. Protected by copyright. http://jmg.bmj.com/ J Med Genet: first published as 10.1136/jmedgenet-2015-103233 on 14 January 2016. Downloaded from Pierpont syndrome (Z Stark, M Decamp, B Dallapiccola, personal communications, 2014). In one additional published patient the phenotype was attributed to a 2.2 Mb deletion at 3q26.3 involving TBL1XR1, but an updated annotation showed that the deletion did not encompass TBL1XR1.31 This suggests that the phenotype of individuals with a microdeletion 3q26.3 is caused by a loss of function of TBL1XR1, and consists of intellectual disability and frequently ASD, and a phenotype that shows no resemblance to Pierpont syndrome. It remains at present uncertain whether the abundant hypothalamic and pituitary mRNA expression of wild-type TBL1XR1 reported here is related to the intellectual disability in individuals with deletions. The differences in phenotype in subjects with the Y446C mutation compared with subjects with whole-gene deletions or other TBL1XR1 mutations suggest different pathogenic mechanisms. The Y446C mutation could act in a dominant negative way, in agreement with our finding that the mutant TBL1XR1 Y446C protein is able to assemble into the HDAC3 corepressor complex. Since TBL1XR1 Y446C can form hetero-tetramers with wild-type TBL1XR1, TBL1XR1 Y446C will likely be present in most HDAC3 corepressor complexes, and the impaired or inappropriate protein–protein interactions with an as yet unidentified molecular partner will likely be present ubiquitously.
In conclusion, one specific TBL1XR1 missense mutation is responsible for the phenotype in individuals with Pierpont syndrome.
The difference in phenotype between non-ASD Pierpont patients with the Y446C mutation in TBL1XR1 and individuals with a complete deletion or other mutation of TBL1XR1 suggests mutation-specific mechanisms of pathogenesis for ASD. Further studies of the pathogenesis in individuals with deletions and various mutations in TBL1XR1 could yield useful insights into the pathogenesis of ASD.
Author affiliations
1 Department of Endocrinology and Metabolism, Academic Medical Centre, University of Amsterdam, Amsterdam, The Netherlands 2 Department of Paediatric Endocrinology, Emma Children’s Hospital, Academic Medical Centre, University of Amsterdam, Amsterdam, The Netherlands
3 Department of Clinical Epidemiology, Biostatistics and Bioinformatics, Academic Medical Centre, University of Amsterdam, Amsterdam, The Netherlands
4 Department of Biochemistry, Henry Wellcome Laboratories of Structural Biology, University of Leicester, Leicester, UK
5 Department of Clinical Genetics, Academic Medical Centre, University of Amsterdam, Amsterdam, The Netherlands
6 Medical Genetics Unit, Ospedali Galliera, Genova, Italy
7 Department of Genetics, University of Groningen, University Medical Centre Groningen, Groningen, The Netherlands
8 Division of Metabolism, Kennedy Krieger Institute, Johns Hopkins University, Baltimore, Maryland, USA
9 Munroe-Meyer Institute for Genetics and Rehabilitation, University of Nebraska Medical Centre, Omaha, Nebraska, USA
10 Division of Genetics, Children’s Hospitals and Clinics of Minnesota, University of Minnesota, Minneapolis, Minnesota, USA
11 Division of Medical Genetics, Arkansas Children’s Hospital, Little Rock, Arkansas, USA
12 Division of Medical Genetics, Belfast City Hospital, Belfast, Ireland
13 Department of Paediatrics, Emma Children’s Hospital, Academic Medical Centre, University of Amsterdam, Amsterdam, The Netherlands Acknowledgements We thank the patients and their families who participated in the study. We are indebted to Drs B O’Roak, E Eichler, Z Stark, M Decamp and B Dallapiccola for their kind help in collecting phenotypic data from cases reported in the literature or in Decipher. We also thank Dr A H van der Spek for providing whole blood samples from healthy controls, Drs M Serlie, P Gilijamse and M Kilicarslan for providing cDNA of liver biopsies and white adipose tissue biopsies and Drs E Nascimento, E Broeders, N Bouvy, P Schrauwen and W van Marken Lichtenbelt for providing biopsies of brown adipose tissue. We thank the help of the Galliera Genetic Bank, member of the Telethon Genetic Biobank Network (Project No. GTB12001), funded by Telethon Italy. Contributors CAH and RCH collected and analysed data, and wrote the manuscript. AJ, BR and RCH performed exome sequencing data validation and mutation analysis. PJW, AB, OB-S and JWRS performed functional studies. FF, RH, RK, AHO, MEP, GBS, FS, ASPvT and EF collected patient samples and analysed data. RCH conceived the project and supervised the experiments. All authors revised the manuscript critically and approved the final version for publication. Funding This work was financially supported by an AMC Foundation grant. JWRS is supported by a Senior Investigator Award WT100237 from the Wellcome Trust and a Biotechnology and Biological Sciences Research Council Project Grant BB/ J009598/1. JWRS is a Royal Society Wolfson Research Merit Award Holder. Patient consent Obtained. Ethics approval Medical Ethics Committee of the Academic Medical Centre in Amsterdam. Provenance and peer review Not commissioned; externally peer reviewed. Open Access This is an Open Access article distributed in accordance with the terms of the Creative Commons Attribution (CC BY 4.0) license, which permits others to distribute, remix, adapt and build upon this work, for commercial use, provided the original work is properly cited. See: http://creativecommons.org/ licenses/by/4.0/
REFERENCES
1 Pierpont M, Stewart F, Gorlin R. Plantar lipomatosis, unusual facial phenotype and developmental delay: a new MCA/MR syndrome. Am J Med Genet 1998;75:18–21.
2 Oudesluijs GG, Hordijk R, Boon M, Sijens PE, Hennekam RC. Plantar lipomatosis, unusual facies, and developmental delay: confirmation of Pierpont syndrome. Am J Med Genet 2005;137A:77–80.
3 Vadivelu S, Edelman M, Schneider SJ, Mittler MA. Choroid plexus papilloma and Pierpont syndrome. J Neurosurg Pediatr 2013;11:115–8.
4 Wright EM, Suri M, White SM, de Leeuw N, Vulto-van Silfhout AT, Stewart F, McKee S, Mansour S, Connell FC, Chopra M, Kirk EP, Devriendt K, Reardon W, Brunner H, Donnai D. Pierpont syndrome: a collaborative study. Am J Med Genet 2011;155A:2203–11.
5 Sim JC, White SM, Fitzpatrick E, Wilson GR, Gillies G, Pope K, Mountford HS, Torring PM, McKee S, Vulto-van Silfhout AT, Jhangiani SN, Muzny DM, Leventer RJ, Delatycki MB, Amor DJ, Lockhart PJ. Expanding the phenotypic spectrum of ARID1B-mediated disorders and identification of altered cell-cycle dynamics due to ARID1B haploinsufficiency. Orphanet J Rare Dis 2014;9:43.
6 McKenna A, Hanna M, Banks E, Sivachenko A, Cibulskis K, Kernytsky A, Garimella K, Altshuler D, Gabriel S, Daly M, DePristo MA. The Genome Analysis Toolkit: a MapReduce framework for analyzing next-generation DNA sequencing data. Genome Res 2010;20:1297–303.
7 DePristo MA, Banks E, Poplin R, Garimella KV, Maguire JR, Hartl C, Philippakis AA, del Angel G, Rivas MA, Hanna M, McKenna A, Fennell TJ, Kernytsky AM, Sivachenko AY, Cibulskis K, Gabriel SB, Altshuler D, Daly MJ. A framework for variation discovery and genotyping using next-generation DNA sequencing data. Nat Genet 2011;43:491–8.
8 Li MX, Gui HS, Kwan JS, Bao SY, Sham PC. A comprehensive framework for prioritizing variants in exome sequencing studies of Mendelian diseases. Nucleic Acids Res 2012;40:e53.
9 Watson PJ, Fairall L, Santos GM, Schwabe JW. Structure of HDAC3 bound to co-repressor and inositol tetraphosphate. Nature 2012;481:335–40.
10 Bisschop PH, Dekker MJ, Osterthun W, Kwakkel J, Anink JJ, Boelen A, Unmehopa UA, Koper JW, Lamberts SW, Stewart PM, Swaab DF, Fliers E. Expression of 11beta-hydroxysteroid dehydrogenase type 1 in the human hypothalamus. J Neuroendocrinol 2013;25:425–32.
11 Ramakers C, Ruijter JM, Deprez RH, Moorman AF. Assumption-free analysis of quantitative real-time polymerase chain reaction (PCR) data. Neurosci Lett 2003;339:62–6.
12 Cerna D, Wilson DK. The structure of Sif2p, a WD repeat protein functioning in the SET3 corepressor complex. J Mol Biol 2005;351:923–35.
13 Zhang XM, Chang Q, Zeng L, Gu J, Brown S, Basch RS. TBLR1 regulates the expression of nuclear hormone receptor co-repressors. BMC Cell Biol 2006;7:31.
14 Oberoi J, Fairall L, Watson PJ, Yang JC, Czimmerer Z, Kampmann T, Goult BT, Greenwood JA, Gooch JT, Kallenberger BC, Nagy L, Neuhaus D, Schwabe JW. Structural basis for the assembly of the SMRT/NCoR core transcriptional repression machinery. Nat Struct Mol Biol 2011;18:177–84.
15 Hu X, Lazar MA. Transcriptional repression by nuclear hormone receptors. Trends Endocrinol Metab 2000;11:6–10.
16 Tagami T, Madison LD, Nagaya T, Jameson JL. Nuclear receptor corepressors activate rather than suppress basal transcription of genes that are negatively regulated by thyroid hormone. Mol Cell Biol 1997;17:2642–8.
17 Perissi V, Aggarwal A, Glass CK, Rose DW, Rosenfeld MG. A corepressor/coactivator exchange complex required for transcriptional activation by nuclear receptors and other regulated transcription factors. Cell 2004;116:511–26. 336 Heinen CA, et al. J Med Genet 2016;53:330–337. doi:10.1136/jmedgenet-2015-103233 Developmental defects on February 17, 2021 by guest. Protected by copyright. http://jmg.bmj.com/ J Med Genet: first published as 10.1136/jmedgenet-2015-103233 on 14 January 2016. Downloaded from
18 Hoberg JE, Yeung F, Mayo MW. SMRT derepression by the IkappaB kinase alpha: a prerequisite to NF-kappaB transcription and survival. Mol Cell 2004;16:245–55.
19 Li J, Wang CY. TBL1-TBLR1 and beta-catenin recruit each other to Wnt target-gene promoter for transcription activation and oncogenesis. Nat Cell Biol 2008;10:160–9.
20 Braggio E, McPhail ER, Macon W, Lopes MB, Schiff D, Law M, Fink S, Sprau D, Giannini C, Dogan A, Fonseca R, O’Neill BP. Primary central nervous system lymphomas: a validation study of array-based comparative genomic hybridization in formalin-fixed paraffin-embedded tumor specimens. Clin Cancer Res 2011;17:4245–53.
21 Gonzalez-Aguilar A, Idbaih A, Boisselier B, Habbita N, Rossetto M, Laurenge A, Bruno A, Jouvet A, Polivka M, Adam C, Figarella-Branger D, Miquel C, Vital A, Ghesquières H, Gressin R, Delwail V, Taillandier L, Chinot O, Soubeyran P, Gyan E, Choquet S, Houillier C, Soussain C, Tanguy ML, Marie Y, Mokhtari K, Hoang-Xuan K. Recurrent mutations of MYD88 and TBL1XR1 in primary central nervous system lymphomas. Clin Cancer Res 2012;18:5203–11.
22 Parker H, An Q, Barber K, Case M, Davies T, Konn Z, Stewart A, Wright S, Griffiths M, Ross FM, Moorman AV, Hall AG, Irving JA, Harrison CJ, Strefford JC. The complex genomic profile of ETV6-RUNX1 positive acute lymphoblastic leukemia highlights a recurrent deletion of TBL1XR1. Genes Chromosomes Cancer 2008;47:1118–25.
23 Andersson E, Eldfors S, Edgren H, Ellonen P, Väkevä L, Ranki A, Mustjoki S. Novel TBL1XR1, EPHA7 and SLFN12 mutations in a Sezary syndrome patient discovered by whole exome sequencing. Exp Dermatol 2014;23:366–8.
24 Liu L, Lin C, Liang W, Wu S, Liu A, Wu J, Zhang X, Ren P, Li M, Song L. TBL1XR1 promotes lymphangiogenesis and lymphatic metastasis in esophageal squamous cell carcinoma. Gut 2015;64:26–36.
25 Kadota M, Sato M, Duncan B, Ooshima A, Yang HH, Diaz-Meyer N, Gere S, Kageyama S, Fukuoka J, Nagata T, Tsukada K, Dunn BK, Wakefield LM, Lee MP. Identification of novel gene amplifications in breast cancer and coexistence of gene amplification with an activating mutation of PIK3CA. Cancer Res 2009;69:7357–65.
26 O’Roak BJ, Vives L, Girirajan S, Karakoc E, Krumm N, Coe BP, Levy R, Ko A, Lee C, Smith JD, Turner EH, Stanaway IB, Vernot B, Malig M, Baker C, Reilly B, Akey JM, Borenstein E, Rieder MJ, Nickerson DA, Bernier R, Shendure J, Eichler EE. Sporadic autism exomes reveal a highly interconnected protein network of de novo mutations. Nature 2012;485:246–50.
27 O’Roak BJ, Vives L, Fu W, Egertson JD, Stanaway IB, Phelps IG, Carvill G, Kumar A, Lee C, Ankenman K, Munson J, Hiatt JB, Turner EH, Levy R, O’Day DR, Krumm N, Coe BP, Martin BK, Borenstein E, Nickerson DA, Mefford HC, Doherty D, Akey JM, Bernier R, Eichler EE, Shendure J. Multiplex targeted sequencing identifies recurrently mutated genes in autism spectrum disorders. Science 2012;338:1619–22.
28 Saitsu H, Tohyama J, Walsh T, Kato M, Kobayashi Y, Lee M, Tsurusaki Y, Miyake N, Goto Y, Nishino I, Ohtake A, King MC, Matsumoto N. A girl with West syndrome and autistic features harboring a de novo TBL1XR1 mutation. J Hum Genet 2014;59:581–3.
29 Tabet AC, Leroy C, Dupont C, Serrano E, Hernandez K, Gallard J, Pouvreau N, Gadisseux JF, Benzacken B, Verloes A. De novo deletion of TBL1XR1 in a child with non-specific developmental delay supports its implication in intellectual disability. Am J Med Genet 2014;164A:2335–7.
30 Pons L, Cordier MP, Labalme A, Till M, Louvrier C, Schluth-Bolard C, Lesca G, Edery P, Sanlaville D. A new syndrome of intellectual disability with dysmorphism due to TBL1XR1 deletion. Am J Med Genet A 2015;167A:164–8.
31 Millson A, Lagrave D, Willis MJ, Rowe LR, Lyon E, South ST. Chromosomal loss of 3q26.3–3q26.32, involving a partial neuroligin 1 deletion, identified by genomic microarray in a child with microcephaly, seizure disorder, and severe intellectual disability. Am J Med Genet 2012;158A:159–65. Heinen CA, et al. J Med Genet 2016;53:330–337. doi:10.1136/jmedgenet-2015-103233 337 Developmental defects on February 17, 2021 by guest. Protected by copyright. http://jmg.bmj.com/ J Med Genet: first published as 10.1136/jmedgenet-2015-103233 on 14 January 2016.
Downloaded from Correction Heinen C, Jongejan A, Watson PJ, et al. A specific mutation in TBL1XR1 causes Pierpont syndrome. J Med Genet 2016;53:330–7 doi:10.1136/jmedgenet-2015-103233. In the results section of the abstract and the molecular analysis of the results section the mutation in TBL1XR1 was reported as c.1337A>C. The correct notation is c.1337A>G. J Med Genet 2016;53:430. doi:10.1136/jmedgenet-2015-103233corr1 430 Aldinger KA, et al. J Med Genet 2016;53:427–430. doi:10.1136/jmedgenet-2015-103476
TBL1XR1 is a gene, located on chromosome 3q26.32, that is present in all humans.
Genes contain instructions to make proteins. Proteins perform many critical roles in the body, including maintaining the structure, function, and regulation of tissues and organs.
Research indicates that the TBL1XR1 gene plays an important role in human development, in particular for brain function. TBL1XR1 also plays an important role in regulating gene pathways (turning various genes “on” and “off”).
Articles on TBL1XR1 gene:
Expanding the spectrum of TBL1XR1 deletion: Report of a patient with brain and cardiac malformations, European Journal of Medical Genetics (2018): https://www.sciencedirect.com/science/article/pii/S1769721216304943
A specific mutation in TBL1XR1 causes Pierpont syndrome, Journal of Medical Genetics (2015): https://jmg.bmj.com/content/53/5/330
Linkage between TBL1XR1 and autism: https://www.the-scientist.com/features/the-genes-underlying-autism-are-coming-into-focus-33113 (must create login, but free to read).
TBL1XR1 in physiological and pathological states, American Journal of Clinical and Experimental Urology (2015): https://www.ncbi.nlm.nih.gov/pmc/articles/PMC4446378/
Although the effect of having a TBL1XR1 gene variant can vary greatly from one person to another, common symptoms include:
Intellectual and Developmental Disabilities
Epilepsy (Seizures)
Autism Spectrum Disorder
ADHD
Chiari Malformation (brain)
Heart Defects
Kidney Defects
Strabismus (vision)
Feeding Intolerance
Hypotonia (low muscle tone)
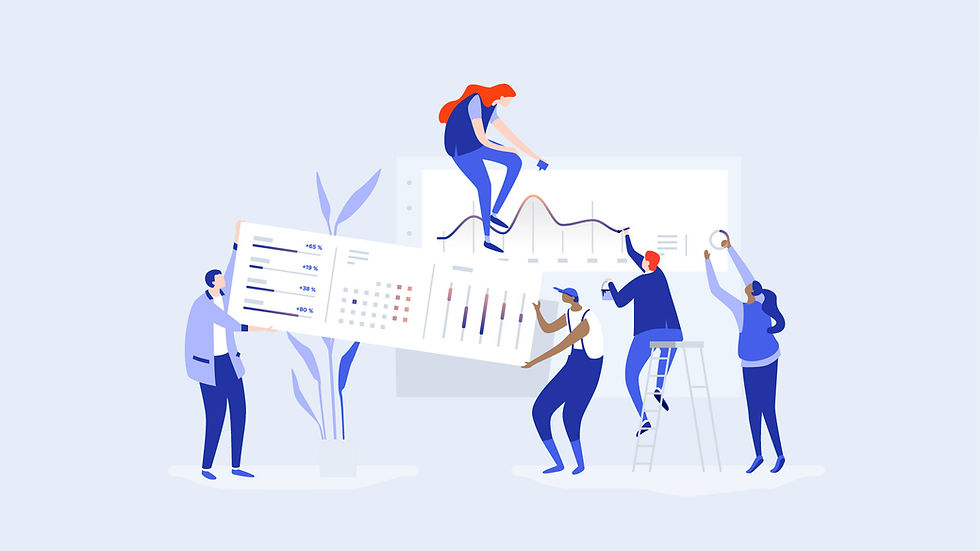
Comentarios